Why do we need to know what the resting potential is?
What is "animal electricity"? Where do bio-currents come from in the body? How can a living cell in an aquatic environment turn into an "electric battery"?
- We can answer these questions if we find out how a cell creates an electric potential on the membrane due to the redistribution of electric charges (charged particles - ions) .
How does the nervous system work? Where does it all begin? Where does the electricity for nerve impulses come from?
- We can also answer these questions if we find out how a nerve cell creates an electric potential for itself on the membrane.
So, understanding how the nervous system works begins with understanding how a single nerve cell, a neuron, works.
The work of a neuron with nerve impulses is based on the redistribution of electrical charges on its membrane and a change in the magnitude of electrical potentials. But in order for the potential to change, you must first have it. Therefore, we can say that a neuron, preparing for its nervous work, creates an electrical potential on its membrane as an opportunity for such work.
Thus, our very first step towards studying the functioning of the nervous system is to understand how electrical charges move in nerve cells and how this creates an electrical potential on the membrane. This is what we will do, and we will call this process of the appearance of electric potential in neurons - the formation of resting potential.
Definition
Normally, when a cell is ready to work, it already has an electrical potential on the membrane surface. It is called the resting membrane potential.
The resting potential is the difference in electrical potential between the inner and outer sides of the membrane when the cell is in a state of physiological rest. Its average value is -70 mV (millivolts).
"Potential" is an opportunity, it is akin to the concept of "potency". The electrical potential of a membrane is its ability to move electrical charges, positive or negative. In the role of charges are charged chemical particles - sodium and potassium ions, as well as calcium and chlorine. Of these, only chloride ions are negatively charged (-), while the rest are positively charged (+).
Table 1. Physicochemical properties of sodium and potassium ions

The table shows that sodium and potassium in the ionized state do not differ from each other in charge, differ little in size and number of coordination bonds they create, but differ significantly in the limiting temperature, that is, the temperature above which their hydration is allowed (T before). For sodium it is +20°C, and for potassium +70°C. Thus, at least in the temperature range above +20°C, in which most living organisms function, sodium easily interacts with water molecules, forming a hydration shell, and potassium repels water. Since the hydrated sodium ion is close in size to the non-hydrated potassium ion, in aqueous solutions these ions do not differ from each other either in charge or in size, and the most significant difference for distinguishing them is the hydrophobicity value. Quantitatively, this value can be expressed by the energy of hydration, which at room temperature is +1.03 kJ/mol for sodium, and -1.05 kJ/mol for potassium. Obviously, biological molecules can use this parameter to select and recognize these two ions. In particular,+ and K + , probably due to differences in their hydrophobicity. If, for example, vesicle vesicles are prepared from a mixture of natural lipids (such a simplified prototype of cell vesicles is called liposomes ), then it turns out that the rate of simple diffusion through their membranes will be 3–7 times higher for potassium than for sodium (depending on the composition lipids, ionic strength and other conditions). So under normal conditions, it is always more difficult for sodium than for potassium to move through the cell membrane. And the cell, accordingly, is easier to restrain the movement of sodium through its membrane than potassium. Potassium is unstoppable!
However, both Na + and K + are potentially able to move under the influence of electric forces to where not positive, but negative electric charges prevail (negatively charged particles are anions).
So, having an electrical potential, the membrane can move the above charged ions into or out of the cell with the help of electrical forces.
It is important to understand that in the nervous system, electric charges are created not by electrons, as in metal wires, but by ions - chemical particles that have an electric charge. The electric current in the body and its cells is a flow of ions, not electrons, as in wires. Also note that the membrane charge is measured from the inside of the cell, not the outside.
Speaking quite primitively simply, it turns out that “pluses” will prevail outside around the cell, i.e. positively charged ions, and inside - "minus signs", i.e. negatively charged ions. We can say that inside the cell is electronegative. And now we just need to explain how it happened. Although, of course, it is unpleasant to realize that all our cells are negative "characters".
Essence
The essence of the resting potential is the predominance of negative electric charges in the form of anions on the inside of the membrane and the lack of positive electric charges in the form of cations, which are concentrated on its outside, and not on the inside.
Inside the cell - "negativity", and outside - "positivity".
This state of affairs is achieved through three phenomena:
- the behavior of the membrane,
- the behavior of positive potassium and sodium ions, and
- the relationship between chemical and electrical force.
1. Membrane behavior
Three processes are important in the behavior of the membrane for the resting potential:
- Exchange of internal sodium ions for external potassium ions. The exchange is carried out by special membrane transport structures: ion exchanger pumps. In this way, the membrane oversaturates the cell with potassium but depletes it with sodium.
- Open potassium ion channels. Through them, potassium can both enter the cell and leave it. He goes out basically.
- Closed sodium ion channels. Because of this, sodium removed from the cell by exchange pumps cannot return to it. Sodium channels open only under special conditions - and then the resting potential is disturbed and shifted towards zero (this is called membrane depolarization, i.e. a decrease in polarity).
2. Behavior of potassium and sodium ions
Potassium and sodium ions move across the membrane in different ways:
- Through ion exchange pumps, sodium is forcibly removed from the cell, and potassium is dragged into the cell.
- Through constantly open potassium channels, potassium leaves the cell, but can also return to it back through them.
- Sodium "wants" to enter the cell, but "can't", because channels are closed to him.
3. The ratio of chemical and electrical forces
In relation to potassium ions, a balance is established between the chemical and electrical forces at a level of - 70 mV.
- The chemical force pushes potassium out of the cell but tends to pull sodium into it.
- The electrical force tends to draw any positively charged ions (both sodium and potassium) into the cell.
Resting potential formation
I will try to tell you briefly where the resting membrane potential comes from in nerve cells - neurons. After all, as everyone now knows, our cells are only positive on the outside, but inside they are very negative, and in them, there is an excess of negative particles - anions and a lack of positive particles - cations.
And here one of the logical traps awaits the researcher and student: the internal electronegativity of the cell does not arise due to the appearance of extra negative particles (anions), but, on the contrary, due to the loss of a certain amount of positive particles (cations).
And therefore, the essence of our story will not be that we will explain where the negative particles come from in the cell, but that we will explain how the deficit of positively charged ions - cations - is obtained in neurons.
Where do the positively charged particles go from the cell? Let me remind you that these are sodium ions - Na + and potassium - K + . Surprisingly, both of these ions leave the cell, albeit in different ways.
Where to start, so as not to stray and not get confused?
Let's start with potassium.
To make it easier to remember, figuratively, you can say this:
"The cell loves potassium!"
or: "the cell accumulates potassium!" (K + )
and "hates sodium" (Na + ).
And what does she, the cell, do with these ions? She trades them for each other! With the help of a special exchange pump.
Sodium-potassium pump
The point is that exchanger pumps, formed by special proteins built into the membrane, are constantly working in the membrane of the nerve cell. What are they doing? They change the "own" sodium of the cell to the external "foreign" potassium. Because of this, the cell ends up with a lack of sodium, which has gone to the exchange. And at the same time, the cell is overflowing with potassium ions, which these molecular pumps have dragged into it.
So: " The cell loves potassium! " (Although true love is, of course, out of the question here!) Therefore, it drags potassium into itself, despite the fact that it is already full of it. Therefore, she unprofitably exchanges it for sodium, giving 3 sodium ions for 2 potassium ions (beloved). Therefore, it spends ATP energy on this exchange. And how to spend! Up to 70% of all neuron energy consumption can be spent on the work of sodium-potassium pumps. That's what love does, even if it's not real!
By the way, it is interesting that a cell is not born with a resting potential in its finished form. For example, during differentiation and fusion of myoblasts during the formation of a muscle fiber, the potential of their membrane changes from -10 to -70 mV, i.e. their membrane becomes more electronegative, it becomes polarized during differentiation. And in experiments on multipotent mesenchymal stromal cells (MMSC) of human bone marrow, artificial depolarization inhibited differentiation клеток (Fischer-Lougheed J., Liu J.H., Espinos E. et al. Human myoblast fusion requires expression of functional inward rectifier Kir2.1 channels. Journal of Cell Biology 2001; 153: 677-85; Liu J.H., Bijlenga P., Fischer-Lougheed J. et al. Role of an inward rectifier K+ current and of hyperpolarization in human myoblast fusion. Journal of Physiology 1998; 510: 467-76; Sundelacruz S., Levin M., Kaplan D.L. Membrane potential controls adipogenic and osteogenic differentiation of mesenchymal stem cells. Plos One 2008;
3). Figuratively speaking, it can be expressed as follows:
By creating a resting potential, the cell is "charged with love."
It's love for two things:
- cell love for potassium,
- the love of potassium for freedom.
Oddly enough, the result of these two types of love is emptiness!
It is this emptiness that creates a negative electrical potential in the cell - the potential of rest. More precisely, the negative potential is created by empty spaces left from the potassium that escaped from the cell.
So, the result of the activity of membrane ion exchanger pumps is as follows:
The sodium-potassium ion exchange pump creates three potentials (opportunities):
- Electric potential - the ability to attract any positively charged particles (ions) into the cell.
- Ionic sodium chemical potential - the ability to draw sodium ions into the cell (and it is sodium and not some other substance).
- Ionic potassium chemical potential - the ability to push potassium ions out of the cell (and namely potassium, and not some other substance).
- Sodium deficiency (Na + ) in the cell.
- Excess potassium (K + ) in the cell.
We can say this: the ion pumps of the membrane create a difference in ion concentrations, or a concentration gradient (difference), between the intracellular and extracellular environment.
It is because of the resulting sodium deficiency that this very sodium will now "crawl" into the cell from the outside. This is how substances always behave: they tend to equalize their concentration in the entire volume of the solution.
And at the same time, an excess of potassium ions turned out in the cell compared to the external environment. Because the membrane pumps pumped it into the cell. And he seeks to equalize his concentration inside and outside and therefore seeks to get out of the cage.
Here it is also important to understand that sodium and potassium ions, as it were, "do not notice" each other, they react only "to themselves." Those. sodium reacts to the concentration of sodium, but "does not pay attention" to how much potassium is around. Conversely, potassium reacts only to the concentration of potassium and "does not notice" sodium. It turns out that in order to understand the behavior of ions in a cell, it is necessary to separately compare the concentrations of sodium and potassium ions. Those. it is necessary to separately compare the concentration of sodium inside and outside the cell and separately the concentration of potassium inside and outside the cell, but it makes no sense to compare sodium with potassium, as is often done in textbooks.
According to the law of concentration equalization, which operates in solutions, sodium "wants" to enter the cell from the outside. But it cannot, since the membrane in its normal state does not pass it well. It comes in a little, and the cell again immediately exchanges it for external potassium. Therefore, sodium in neurons is always in short supply.
But potassium just can easily get out of the cell! The cage is full of him, and she can't keep him. So it comes out through special protein holes in the membrane (ion channels).
Analysis
From chemical to electrical
And now - the most important thing, follow the stated thought! We must move from the movement of chemical particles to the movement of electric charges.
Potassium is charged with a positive charge, and therefore when it leaves the cell, it takes out of it not only itself but also "pluses" (positive charges). In their place, "minuses" (negative charges) remain in the cell. This is the resting membrane potential!
The resting membrane potential is a deficit of positive charges (positively charged ions) inside the cell, formed due to the leakage of positive potassium ions from the cell.
Conclusion


Components of the resting potential
The resting potential is negative from the side of the cell and consists, as it were, of two parts.
- The first part is approximately -10 millivolts, which are obtained from the non-equilateral operation of the membrane exchanger pump (after all, it pumps out more “pluses” with sodium than it pumps back with potassium).
- The second part is potassium leaking out of the cell all the time, dragging positive charges out of the cell. It gives most of the membrane potential, bringing it down to -70 millivolts.
- Potassium will stop leaving the cell (more precisely, its input and output will be equal) only when the electronegativity level of the cell is -90 millivolts. But this is hindered by sodium constantly leaking into the cell, which drags its positive charges with it. And the cell maintains an equilibrium state at the level of -70 millivolts.
Note:
It takes energy to create the resting potential. These costs are produced by ion pumps that exchange "own" internal sodium (Na + ions ) for "foreign" external potassium (K +). Recall that ion pumps are ATPase enzymes and break down ATP, obtaining energy from it for the indicated exchange of different types of ions with each other. It is very important to understand that 2 potentials “work” with the membrane at once: chemical (concentration gradient of ions) and electric ( electrical potential difference on opposite sides of the membrane). Ions move in one direction or another under the action of both of these forces, on which energy is spent. In this case, one of the two potentials (chemical or electrical) decreases, while the other increases. Of course, if we consider the electric potential (potential difference) separately, then the "chemical" forces that move the ions will not be taken into account. And then there may be an incorrect impression that the energy for the movement of the ion is taken, as it were, from nowhere. But it's not. Both forces must be considered: chemical and electrical. In this case, large molecules with negative charges located inside the cell play the role of "extras", because they are not moved across the membrane by either chemical or electrical forces. Therefore, these negative particles are usually not considered, although they exist and it is they that provide the negative side of the potential difference between the inner and outer sides of the membrane. But nimble potassium ions are just capable of moving, and it is their leakage from the cell under the influence of chemical forces that creates the lion's share of the electrical potential (potential difference). After all, it is potassium ions that move positive electric charges to the outer side of the membrane, being positively charged particles. In this case, large molecules with negative charges located inside the cell play the role of "extras", because they are not moved across the membrane by either chemical or electrical forces. Therefore, these negative particles are usually not considered, although they exist and it is they that provide the negative side of the potential difference between the inner and outer sides of the membrane. But nimble potassium ions are just capable of moving, and it is their leakage from the cell under the influence of chemical forces that creates the lion's share of the electrical potential (potential difference). After all, it is potassium ions that move positive electric charges to the outer side of the membrane, being positively charged particles. In this case, large molecules with negative charges located inside the cell play the role of "extras", because they are not moved across the membrane by either chemical or electrical forces. Therefore, these negative particles are usually not considered, although they exist and it is they that provide the negative side of the potential difference between the inner and outer sides of the membrane. But nimble potassium ions are just capable of moving, and it is their leakage from the cell under the influence of chemical forces that creates the lion's share of the electrical potential (potential difference). After all, it is potassium ions that move positive electric charges to the outer side of the membrane, being positively charged particles. nor electrical power. Therefore, these negative particles are usually not considered, although they exist and it is they that provide the negative side of the potential difference between the inner and outer sides of the membrane. But nimble potassium ions are just capable of moving, and it is their leakage from the cell under the influence of chemical forces that creates the lion's share of the electrical potential (potential difference). After all, it is potassium ions that move positive electric charges to the outer side of the membrane, being positively charged particles. nor electrical power. Therefore, these negative particles are usually not considered, although they exist and it is they that provide the negative side of the potential difference between the inner and outer sides of the membrane. But nimble potassium ions are just capable of moving, and it is their leakage from the cell under the influence of chemical forces that creates the lion's share of the electrical potential (potential difference). After all, it is potassium ions that move positive electric charges to the outer side of the membrane, being positively charged particles. and it is their leakage from the cell under the action of chemical forces that creates the lion's share of the electrical potential (potential difference). After all, it is potassium ions that move positive electric charges to the outer side of the membrane, being positively charged particles. and it is their leakage from the cell under the action of chemical forces that creates the lion's share of the electrical potential (potential difference). After all, it is potassium ions that move positive electric charges to the outer side of the membrane, being positively charged particles.
So it's all about the sodium-potassium membrane pump-exchanger and the subsequent outflow of "excess" potassium from the cell. Due to the loss of positive charges during this outflow, electronegativity increases inside the cell. It is this "membrane resting potential". It is measured inside the cell and is usually -70 mV.
Conclusions
Figuratively speaking, "the membrane turns the cell into an" electric battery "by controlling ion flows."
The resting membrane potential is formed due to two processes:
1. Operation of the sodium-potassium membrane pump.
A new hypothesis for the mechanism of Na,K-ATPase is discussed here: Mechanism of the sodium-potassium pump
- The work of the potassium-sodium pump, in turn, has 2 consequences:
- Direct electrogenic (generating electrical phenomena) action of the ion exchanger pump. This is the creation of a small electronegativity inside the cell (-10 mV).
- The unequal exchange of sodium for potassium is to blame for this. More sodium is expelled from the cell than potassium is metabolized. And along with sodium, more "pluses" (positive charges) are removed than are returned with potassium. There is a small deficit of positive charges. The membrane is negatively charged from the inside (approximately -10 mV).
- Creation of prerequisites for the emergence of large electronegativity.
These prerequisites are an unequal concentration of potassium ions inside and outside the cell. Excess potassium is ready to leave the cell and carry positive charges out of it. We will talk about this below.
2. Leakage of potassium ions from the cell.
From the zone of increased concentration inside the cell, potassium ions go to the zone of low concentration outside, at the same time carrying positive electrical charges. There is a strong deficit of positive charges inside the cell. As a result, the membrane is additionally charged negatively from the inside (up to -70 mV).
Final
So:
The potassium-sodium pump creates the prerequisites for the emergence of the resting potential. This is the difference in ion concentration between the inside and outside of the cell. Separately, the difference in concentration for sodium and the difference in concentration for potassium manifest themselves. The cell's attempt to equalize the concentration of ions with potassium leads to a loss of potassium, a loss of positive charges, and generates electronegativity within the cell. This electronegativity makes up most of the resting potential. A smaller part of it is the direct electrogenicity of the ion pump, i.e. the predominant loss of sodium during its exchange for potassium.
Formation of the resting membrane potential
The resting potential is an important phenomenon in the life of all cells of the body, and it is important to know how it is formed. However, this is a complex dynamic process, difficult to understand as a whole, especially for undergraduate students (biological, medical and psychological specialties) and unprepared readers. However, when considered point by point, it is quite possible to understand its main details and stages. The paper introduces the concept of the resting potential and highlights the main stages of its formation using figurative metaphors that help to understand and remember the molecular mechanisms of the formation of the resting potential.
Membrane transport structures - sodium-potassium pumps - create the prerequisites for the emergence of resting potential. These prerequisites are the difference in the concentration of ions on the inner and outer sides of the cell membrane. Separately, the difference in concentration for sodium and the difference in concentration for potassium manifest themselves. An attempt of potassium ions (K + ) to equalize their concentration on both sides of the membrane leads to its leakage from the cell and the loss of positive electric charges along with them, due to which the overall negative charge of the inner surface of the cell is significantly increased. This "potassium" negativity makes up most of the resting potential (−60 mV on average), and the smaller part (−10 mV) is the "exchange" negativity caused by the electrogenicity of the ion exchange pump itself.
Resting potential and action potential
The membrane of all living cells is polarized. The inner side of the membrane carries a negative charge compared to the intercellular space (Fig. 1). The amount of charge that a membrane carries is called the membrane potential (MP). In non-excitable tissues, the MP is low and is about -40 mV. In excitable tissues, it is high, about -60 - -100 mV, and is called the resting potential (RP).
The resting potential, like any membrane potential, is formed due to the selective permeability of the cell membrane. As is known, the plasmalemma consists of a lipid bilayer through which the movement of charged molecules is hindered. Proteins embedded in the membrane can selectively change the permeability of the membrane to various ions, depending on incoming stimuli. At the same time, potassium ions play a leading role in the formation of the resting potential, in addition to them, sodium and chlorine ions are important.
Fig. 1
Concentrations and distribution of ions from the inner and outer sides of the membrane
Most of the ions are distributed unevenly from the inside and outside of the cell (Fig. 1). Inside the cell, the concentration of potassium ions is higher, and sodium and chlorine are lower than outside. At rest, the membrane is permeable to potassium ions and practically impermeable to sodium and chloride ions. Despite the fact that potassium can freely leave the cell, its concentrations remain unchanged due to the negative charge on the inside of the membrane. Thus, two forces that are in equilibrium act on potassium: osmotic (K + concentration gradient ) and electrical (membrane charge), due to which the number of potassium ions entering the cell is equal to those leaving. The movement of potassium is carried out through potassium leakage channels open at rest. The value of the membrane charge at which potassium ions are in equilibrium can be calculated using the Nernst equation:
E m = E k = RT / nF ln [K + ] n / [K + ] vn
where E k is the equilibrium potential for K + ; R is the gas constant; T is the absolute temperature; F is the Faraday number; n - valency K + (+1), [K + n ] - [K + ext ] - external and internal concentrations of K + .
If we substitute the values from the table in Fig. 43, then we get the value of the equilibrium potential, equal to approximately -95 mV. This value fits into the range of the membrane potential of excitable cells. Differences in the PP of different cells (even excitable ones) can arise for three reasons:
differences in intracellular and extracellular concentrations of potassium ions in different tissues (the table shows data on the average statistical neuron);
sodium-potassium ATPase can contribute to the charge value since it removes 3 Na + from the cell in exchange for 2 K + ;
despite the minimal permeability of the membrane for sodium and chlorine, these ions can still enter the cells, albeit 10 to 100 times worse than potassium.
To take into account the penetration of other ions into the cell, there is the Nernst-Goldman equation:
Е м = RT / nF ln P k [K + ] вн + P Na [Na + ] вн + P Cl [Cl - ] н / P k [K + ] н + P Na [Na + ] н + P Cl [ Cl - ] vn ,
, where E m is the membrane potential; R is the gas constant; T is the absolute temperature; F is the Faraday number; PK , P Na and P Cl are membrane permeability constants for K + Na + and Cl, respectively; [K + n ], [K + ext ], [Na + n ], [Na + ext ], [Cl - n ] and [Cl - ext ] - concentrations of K + , Na + and Cl outside (n) and inside (ext) of the cell.
This equation allows you to set a more accurate value of the PP. Typically, the membrane is a few mV less polarized than the equilibrium potential for K +.
Action potential (AP) can occur in excitable cells. If a nerve or muscle is irritated above the excitation threshold, then the RI of the nerve or muscle will quickly decrease and for a short period of time (millisecond) there will be a short-term recharge of the membrane: its inner side will become positively charged relative to the outer, after which the RI will be restored. This short-term change in the PP, which occurs when the cell is excited, is called the action potential.
The occurrence of PD is possible due to the fact that, unlike potassium ions, sodium ions are far from equilibrium. If we substitute sodium instead of potassium into the Nernst equation, we get an equilibrium potential of about +60 mV. During PD, there is a transient increase in Na + permeability. At the same time, sodium will begin to penetrate into the cell under the action of two forces: along the concentration gradient and along with the membrane charge, trying to adjust the membrane charge to its equilibrium potential. The movement of sodium is carried out along voltage-dependent sodium channels, which open in response to a shift in the membrane potential, after which they themselves are inactivated.
Fig 2
Fig 3
On the record, the PD looks like a short-term peak, which has several phases.
Depolarization (rising phase) - an increase in sodium permeability due to the opening of sodium channels. Sodium tends to its equilibrium potential but does not reach it, since the channel has time to become inactivated.
Repolarization is the return of the charge to the value of the resting potential. In addition to the potassium channels of the leak, voltage-dependent potassium channels are connected here (activated by depolarization). At this time, potassium leaves the cell, returning to its equilibrium potential.
Hyperpolarization (not always) - occurs in cases where the equilibrium potential for potassium exceeds the modulus of PP. The return to the PP occurs after the return to the equilibrium potential for K + .
During PD, the polarity of the membrane charge changes. The PD phase, in which the membrane charge is positive, is called an overshoot (Fig. 2).
The system of activation and inactivation of voltage-gated sodium channels is very important for AP generation (Fig. 3). These channels have two leaflets: activation (M-gate) and inactivation (H-gate). At rest, the M-gate is open and the H-gate is closed. During membrane depolarization, the M gate opens rapidly and the H gate begins to close. The flow of sodium into the cell is possible while the M-gate is already open, and the H-gate has not yet closed. The entry of sodium leads to further depolarization of the cell, leading to the opening of more channels and starting a positive feedback loop. Membrane depolarization will continue until all voltage-gated sodium channels are inactivated, which occurs at the peak of AP. The minimum value of the stimulus leading to the occurrence of AP is called the threshold. Thus, the emerging AP will obey the all-or-nothing law and its value will not depend on the magnitude of the stimulus that caused the AP.
Due to the H-gate, channel inactivation occurs before the potential on the membrane reaches the equilibrium value for sodium. After the cessation of sodium entry into the cell, repolarization occurs due to potassium ions leaving the cell. At the same time, potential-activated potassium channels are also connected to the leakage channels in this case. During repolarization, the M-gate closes rapidly in the fast sodium channel. The H-gate opens much more slowly and remains closed for some time after the charge returns to the resting potential. This period is called the refractoriness period.
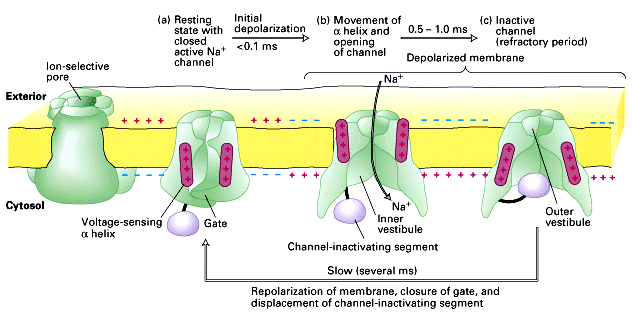
The concentration of ions inside the cell is restored by sodium-potassium ATPase, which, using energy in the form of ATP, pumps 3 sodium ions out of the cell and pumps 2 potassium ions.
The action potential propagates continuously along the unmyelinated fiber or along the muscle membrane. The resulting action potential due to the electric field is able to depolarize the membrane of the neighboring area to a threshold value, resulting in depolarization in the neighboring area. The main role in the emergence of a potential in a new section of the membrane is the previous section. At the same time, at each site, immediately after the AP, a period of refractory occurs, due to which the AP propagates unidirectionally. Ceteris paribus, the propagation of the action potential along the unmyelinated axon occurs the faster, the larger the fiber diameter. In mammals, the speed is 1-4 m / s. Since invertebrates lack myelin, the AP speed in giant squid axons can reach 100 m/s.
By myelinated fiberThe action potential propagates spasmodically (saltatory conduction). Myelinated fibers are characterized by a concentration of voltage-gated ion channels only in the areas of nodes of Ranvier; here their density is 100 times greater than in the membranes of unmyelinated fibers. There are almost no voltage-gated channels in the area of myelin couplings. The action potential that arose in one interception of Ranvier, due to the electric field, depolarizes the membrane of neighboring interceptions to a threshold value, which leads to the emergence of new action potentials in them, that is, excitation passes abruptly, from one interception to another. In the event of damage to one node of Ranvier, the action potential excites the 2nd, 3rd, 4th, and even 5th, since the electrical insulation created by the myelin sleeves reduces the dissipation of the electric field.
The work of neurons
The nervous system is made up of neurons and glial cells. However, the main role in the conduction and transmission of nerve impulses is played by neurons. They receive information from many cells along the dendrites, analyze it and transmit it or not to the next neuron.
The transmission of a nerve impulse from one cell to another is carried out using synapses. There are two main types of synapses: electrical and chemical (Fig. 4). The task of any synapse is to transfer information from the presynaptic membrane (axon membrane) to the postsynaptic membrane (the membrane of a dendrite, another axon, muscle, or other target organ). Most synapses of the nervous system are formed between the end of axons and dendrites, which form dendritic spines in the area of the synapse.
The advantage of an electrical synapse is that the signal from one cell to another passes without delay. In addition, such synapses do not get tired. To do this, pre- and postsynaptic membranes are connected by transverse bridges through which ions from one cell can move to another. However, a significant disadvantage of such a system is the lack of unidirectional transmission of PD. That is, it can be transmitted both from the presynaptic membrane to the postsynaptic one, and vice versa. Therefore, such a design is quite rare and mainly in the nervous system of invertebrates.
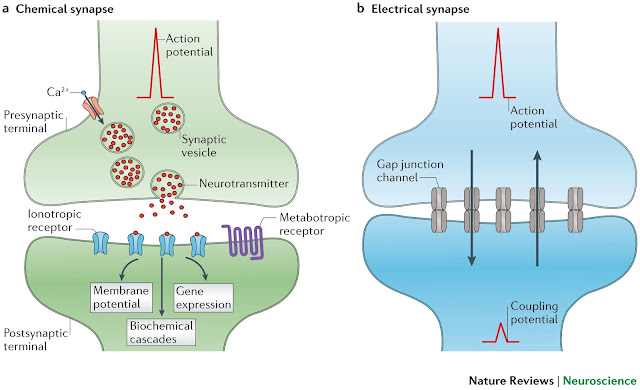
The chemical synapse is very common in nature. О is more complicated, since a system is needed for converting an electrical impulse into a chemical signal, then again into an electrical impulse. All this leads to synaptic delay , which can be 0.2-0.4 ms. In addition, chemical depletion can occur, resulting in synapse fatigue. However, such a synapse provides unidirectional AP transmission, which is its main advantage.
(A)
(B)
Scheme of work (a) and electron micrograph (b) of a chemical synapse
At rest, the axon ending, or presynaptic ending , contains membrane vesicles (vesicles) with a neurotransmitter. The surface of the vesicles is negatively charged to prevent binding to the membrane and is coated with special proteins involved in the release of the vesicles. Each vesicle contains the same amount of a chemical called a neurotransmitter quantum . Neurotransmitters are very diverse in chemical structure, however, most of them are produced right at the end. Therefore, it may contain systems for the synthesis of a chemical mediator, as well as the Golgi apparatus and mitochondria.
The postsynaptic membrane contains receptors for the neurotransmitter. Receptors can be in the form of both ion channels that open upon contact with their ligand ( ionotropic ) and membrane proteins that trigger an intracellular cascade of reactions ( metabotropic ). One neurotransmitter can have several ionotropic and metabotropic receptors. At the same time, some of them can be excitatory, and some - inhibitory. Thus, a cell's response to a neurotransmitter will determine the type of receptor on its membrane, and different cells can respond quite differently to the same chemical.
Between the pre- and postsynaptic membrane is the synaptic cleft , 10-15 nm wide.
When AP arrives at the presynaptic ending, voltage-activated calcium channels open on it and calcium ions enter the cell. Calcium binds to proteins on the surface of the vesicles, which leads to their transport to the presynaptic membrane, followed by membrane fusion. After such an interaction, the neurotransmitter finds itself in the synaptic cleft (Fig. 5) and can bind to its receptor.
Ionotropic receptors are ligand-activated ion channels. This means that the channel only opens in the presence of a certain chemical. For different neurotransmitters, these can be sodium, calcium, or chloride channels. The current of sodium and calcium causes membrane depolarization, therefore such receptors are called excitatory. Chlorine current leads to hyperpolarization, which makes it difficult to generate AP. Therefore, such receptors are called inhibitory.
Metabotropic neurotransmitter receptors belong to the class of G protein-associated receptors (GPCRs). These proteins trigger a variety of intracellular cascades of reactions that ultimately lead to either further transmission of excitation or inhibition.
After signal transmission, it is necessary to quickly remove the neurotransmitter from the synaptic cleft. For this, either enzymes that decompose a neurotransmitter are present in the gap, or transporters pumping the mediator into the cells can be located on the presynaptic ending or neighboring glial cells. In the latter case, it can be reused.
Each neuron receives impulses from 100 to 100,000 synapses. A single depolarization on one dendrite will not result in further signal transmission. A neuron can receive both excitatory and inhibitory stimuli simultaneously. All of them are summarized on the soma of the neuron. Such a summation is called spatial . Further, AP may or may not occur (depending on the signals received) in the region of the axon hillock . The axon hillock is the area of the axon adjacent to the soma and has a minimum AP threshold. Further, the impulse propagates along the axon, the end of which can strongly branch and form synapses with many cells. In addition to spatial summation, there is a temporal summation. It occurs in the case of receipt of frequently repeated impulses from one dendrite.
In addition to classical synapses between axons and dendrites or their spines, there are also synapses that modulate transmission in other synapses (Fig. 6). These include axo-axonal synapses. Such synapses are able to enhance or inhibit synaptic transmission. That is, if an AP arrives at the end of the axon forming the axo-spinous synapse, and at that time an inhibitory signal arrives at the axo-axonal synapse, the release of the neurotransmitter in the axo-spinous synapse will not occur. Axodendritic synapses can change the conduction of AP by the membrane on the way from the spine to the cell soma. There are also axo-somatic synapses that can affect the summation of the signal in the region of the soma of the neuron.
Thus, there is a huge variety of different synapses, differing in the composition of neurotransmitters, receptors and their location. All this provides a variety of reactions and plasticity of the nervous system.
Comments
Post a Comment